Structure of a Muscle
In forming whole muscles, individual muscle fibers are arranged in bundles, or fascicles, held together by fibrous connective tissue (Fig. 4-1). The deepest layer of this connective tissue, the endomysium surrounds the individual fibers in the fascicles. Around each fascicle is a connective tissue layer known as the perimysium. The entire muscle is then encased in a tough connective tissue sheath, the epimysium, which forms the innermost layer of the deep fascia, the tough, fibrous sheath that encloses a muscle. (Note that all these layers are named with prefixes that describe their position added to the root my/o, meaning “muscle.”) All of these supporting tissues merge to form the tendon, the band of connective tissue that attaches a muscle to a bone (see Fig. 4-1).

Figure 4-1 Structure of a skeletal muscle. (A) Structure of a muscle showing the tendon that attaches it to a bone. (B) Muscle tissue seen under a microscope. Portions of several fascicles are shown with connective tissue coverings.
Muscle Cells in Action
Nerve impulses coming from the brain and the spinal cord stimulate skeletal muscle fibers. Because these impulses are traveling away from the central nervous system (CNS), they are described as motor impulses (as contrasted to sensory impulses traveling toward the CNS), and the neurons (nerve cells) that carry these impulses are described as motor neurons. As the neuron contacts the muscle, its axon (fiber) branches to supply from a few to hundreds of individual muscle cells, or in some cases more than 1000 (Fig. 4-2). A single neuron and all the muscle fibers it stimulates comprise a motor unit. Small motor units are used in fine coordination, as in movements of the eye. Larger motor units are used for maintaining posture or for broad movements, such as walking or swinging a tennis racquet.
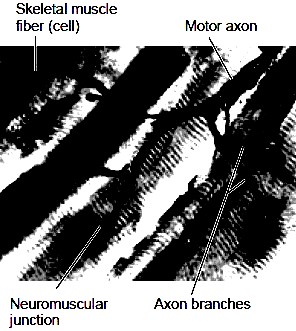
Figure 4-2 Nervous stimulation of skeletal muscle. A motor axon branches to stimulate multiple muscle fibers (cells). The point of contact between the neuron and the muscle cell is the neuromuscular junction.
The Neuromuscular Junction The point at which a nerve fiber contacts a muscle cell is called the neuromuscular junction (NMJ) (Fig. 4-3). It is here that a chemical classified as a neurotransmitter is released from the neuron to stimulate the muscle fiber. The specific neurotransmitter released here is acetylcholine, abbreviated ACh, which is found elsewhere in the body as well. A great deal is known about the events that occur at this junction, and this information is important in understanding muscle action. The neuromuscular junction is an example of a synapse, a point of communication between cells. Between the cells there is a tiny space, the synaptic cleft, across which the neurotransmitter must travel. Until its release, the neurotransmitter is stored in tiny membranous sacs, called vesicles, in the endings of the nerve fiber. Once released, the neurotransmitter crosses the synaptic cleft and attaches to receptors, which are proteins embedded in the muscle cell membrane. The membrane forms multiple folds at this point that increase surface area and hold a maximum number of receptors. The receiving membrane of the muscle cell is known as the motor end plate. Muscle fibers, like nerve cells, show the property of excitability; that is, they are able to transmit electrical current along the plasma membrane. When the muscle is stimulated at the neuromuscular junction, an electrical impulse is generated that spreads rapidly along the muscle cell membrane. This spreading wave of electrical current is called the action potential because it calls the muscle cell into action.

Figure 4-3 Neuromuscular junction (NMJ). (A) The branched end of a motor neuron makes contact with the membrane of a muscle fiber (cell). (B) Enlarged view of the NMJ showing release of neurotransmitter (acetylcholine) into the synaptic cleft. (C) Acetylcholine attaches to receptors in the motor end plate, whose folds increase surface area. (D) Electron microscope photograph of the neuromuscular junction.
Contraction Another important property of muscle tissue is contractility. This is the capacity of a muscle fiber to undergo shortening and to change its shape, becoming thicker. Studies of muscle chemistry and observation of cells under the powerful electron microscope have given a concept of how muscle cells work. These studies reveal that each skeletal muscle fiber contains many threads, or filaments, made of two kinds of proteins, called actin and myosin. Filaments made of actin are thin and light; those made of myosin are thick and dark. The filaments are present in alternating bundles within the muscle cell (Fig. 4-4). It is the alternating bands of light actin and heavy myosin filaments that give skeletal muscle its striated appearance. They also give a view of what occurs when muscles contract. Note that the actin and myosin filaments overlap where they meet, just as your fingers overlap when you fold your hands together. A contracting subunit of skeletal muscle is called a sarcomere. It consists of a band of myosin filaments and the actin filaments on each side of them (see Fig. 4-4). In movement, the myosin filaments “latch on” to the actin filaments in their overlapping region by means of many paddlelike extensions called myosin heads. In this way, the myosin heads form attachments between the actin and myosin filaments that are described as cross-bridges. Using the energy of ATP for repeated movements, the myosin heads, like the oars of a boat moving water, pull all the actin strands closer together within each sarcomere. As the overlapping filaments slide together, the muscle fiber contracts, becoming shorter and thicker. Figure 4-5 shows a section of muscle as it contracts. Once the cross-bridges form, the myosin heads move the actin filaments forward, then they detach and move back to position for another “power stroke.” Note that the filaments overlap increasingly as the cell contracts. (In reality, not all the myosin heads are moving at the same time. About one half are forward at any time, and the rest are preparing for another swing.) During contraction, each sarcomere becomes shorter, but the individual filaments do not change in length. As in shuffling a deck of cards, as you push the cards together, the deck becomes smaller, but the cards do not change in length.

Figure 4-4 Electron microscope photograph of skeletal muscle cell (6500). Actin makes up the light band and myosin makes up the dark band. The dark line in the actin band marks points where actin filaments are held together. A sarcomere is a contracting subunit of skeletal muscle.
Figure 4-5 Sliding filament mechanism of skeletal muscle contraction. (A) Muscle is relaxed and there is no contact between the actin and myosin filaments. (B) Cross-bridges form and the actin filaments are moved closer together as the muscle fiber contracts. (C) The cross-bridges return to their original position and attach to new sites to prepare for another pull on the actin filaments and further contraction.
The Role of Calcium In additional to actin, myosin, and ATP, calcium is needed for muscle contraction. It enables cross-bridges to form between actin and myosin so the sliding filament action can begin. When muscles are at rest, two additional proteins called troponin and tropomyosin block the sites on actin filaments where cross-bridges can form (Fig. 4-6). When calcium attaches to these proteins, they move aside, uncovering the binding sites. In resting muscles, the calcium is not available because it is stored within the endoplasmic reticulum (ER) of the muscle cell. It is released into the cytoplasm only when the cell is stimulated by a nerve fiber. Muscles relax when nervous stimulation stops and the calcium is then pumped back into the ER, ready for the next contraction. A summary of the events in a muscle contraction is as follows:
1. Acetylcholine (ACh) is released from a neuron ending into the synaptic cleft at the neuromuscular junction;
2. ACh binds to the motor end plate of the muscle and produces an action potential;
3. The action potential travels to the endoplasmic reticulum (ER);
4. The endoplasmic reticulum releases calcium into the cytoplasm;
5. Calcium shifts troponin and tropomyosin so that binding sites on actin are exposed;
6. Myosin heads bind to actin, forming cross-bridges;
7. Myosin heads pull actin filaments together within the sarcomeres and cell shortens;
8. ATP is used to detach myosin heads and move them back to position for another “power stroke”;
9. Muscle relaxes when stimulation ends and the calcium is pumped back into the ER.
Figure 4-6 Role of calcium in muscle contraction. (A) Troponin and tropomyosin cover the binding sites where crossbridges can form between actin and myosin. (B) Calcium shifts troponin and tropomyosin away from binding sites so cross-bridges can form.
Energy Sources
As noted earlier, all muscle contraction requires energy in the form of ATP. The source of this energy is the oxidation (commonly called “burning”) of nutrients within the cells. To produce ATP, muscle cells must have an adequate supply of oxygen and glucose or other usable nutrient. The circulating blood constantly brings these substances to the cells, but muscle cells also store a small reserve supply of each to be used when needed, during vigorous exercise, for example. The following are compounds that store oxygen, energy, or nutrients in muscle cells:
* Myoglobin stores additional oxygen. This compound is similar to the blood’s hemoglobin but is located specifically in muscle cells, as indicated by the prefix myo- in its name.
* Glycogen stores additional glucose. It is a polysaccharide made of multiple glucose molecules and it can be broken down into glucose when needed by the muscle cells.
* Creatine phosphate stores energy. It is a compound similar to ATP, in that it has a high energy bond that releases energy when it is broken. This energy is used to make ATP for muscle contraction when the muscle cell has used up its ATP.
Oxygen Consumption During most activities of daily life, the tissues receive adequate oxygen, and muscles can function aerobically. During strenuous activity, however, a person may not be able to breathe in oxygen rapidly enough to meet the needs of the hard-working muscles. At first, the myoglobin, glycogen, and creatine phosphate stored in the tissues meet the increased demands, but continual exercise depletes these stores. For a short time, glucose may be used anaerobically, that is, without the benefit of oxygen. This anaerobic process generates ATP rapidly and permits greater magnitude of activity than would otherwise be possible, as, for example, allowing sprinting instead of jogging. However, anaerobic metabolism is inefficient; it does not produce as much ATP as does metabolism in the presence of oxygen. Also, an organic acid called lactic acid accumulates in the cells when this alternate pathway of metabolism is used. Anaerobic metabolism can continue only until the buildup of lactic acid causes the muscles to fatigue. Muscles operating anaerobically are in a state of oxygen debt. After stopping exercise, a person must continue to take in extra oxygen by continued rapid breathing (panting) until the debt is paid in full. That is, enough oxygen must be taken in to convert the lactic acid to other substances that can be metabolized further. In addition, the glycogen, myoglobin, and creatine phosphate that are stored in the cells must be replenished. The time after strenuous exercise during which extra oxygen is needed is known as the period of recovery oxygen consumption.
Types of Muscle Contractions
Muscle tone refers to a partially contracted state of the muscles that is normal even when the muscles are not in use. The maintenance of this tone, or tonus, is due to the action of the nervous system in keeping the muscles in a constant state of readiness for action. Muscles that are little used soon become flabby, weak, and lacking in tone. In addition to the partial contractions that are responsible for muscle tone, there are two other types of contractions on which the body depends:
* Isotonic contractions are those in which the tone or tension within the muscle remains the same but the muscle as a whole shortens, producing movement; that is, work is accomplished. Lifting weights, walking, running, or any other activity in which the muscles become shorter and thicker (forming bulges) are isotonic contractions.
* Isometric contractions are those in which there is no change in muscle length but there is a great increase in muscle tension. Pushing against an immovable force produces an isometric contraction. For example, if you push the palms of your hands hard against each other, there is no movement, but you can feel the increased tension in your arm muscles. Most movements of the body involve a combination of both isotonic and isometric contractions. When walking, for example, some muscles contract isotonically to propel the body forward, but at the same time, other muscles are contracting isometrically to keep your body in position.